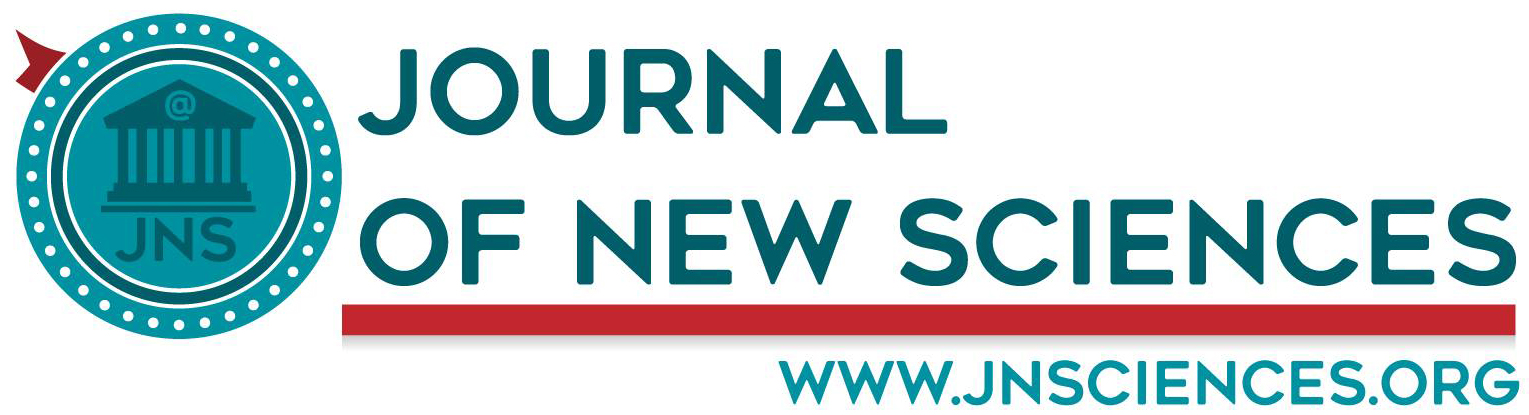
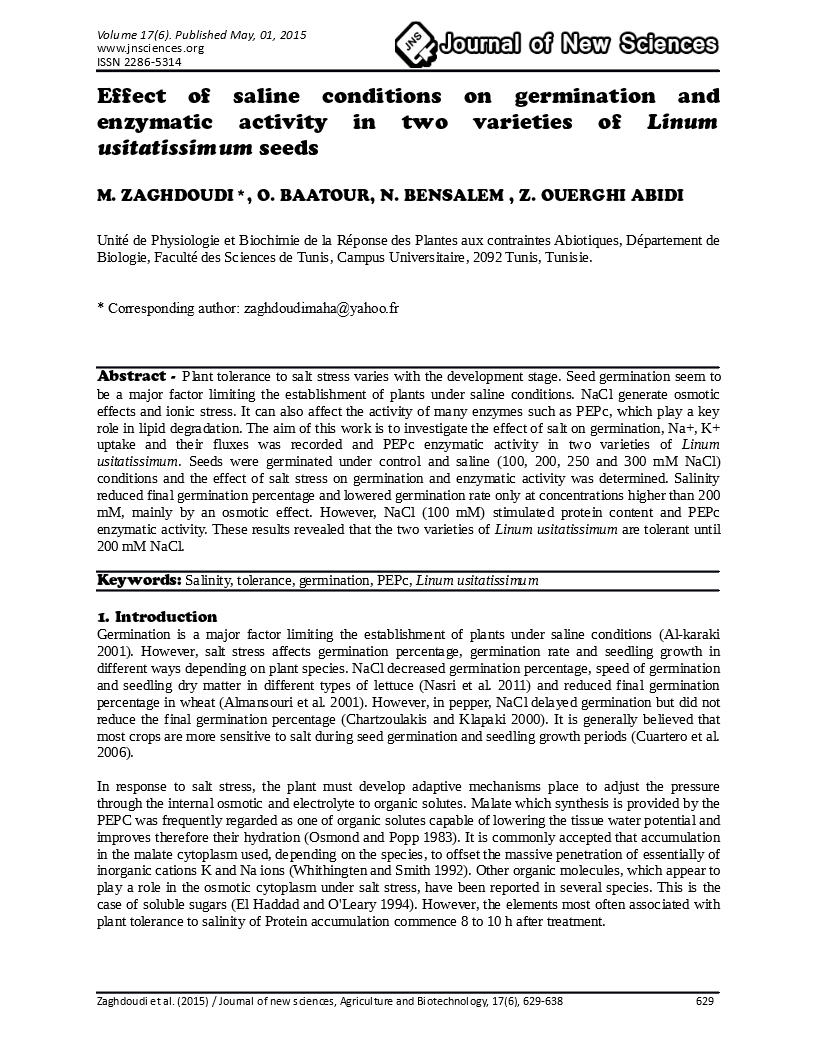
- Category: Volume 17
- Hits: 9804
Effect of saline conditions on germination and enzymatic activity in two varieties of Linum usitatissimum seeds
M. ZAGHDOUDI*
O. BAATOUR
N. BENSALEM
Z. OUERGHI ABIDI
Unité de Physiologie et Biochimie de la Réponse des Plantes aux contraintes Abiotiques, Département de Biologie, Faculté des Sciences de Tunis, Campus Universitaire, 2092 Tunis, Tunisie.
Abstract - Plant tolerance to salt stress varies with the development stage. Seed germination seem to be a major factor limiting the establishment of plants under saline conditions. NaCl generate osmotic effects and ionic stress. It can also affect the activity of many enzymes such as PEPc, which play a key role in lipid degradation. The aim of this work is to investigate the effect of salt on germination, Na+, K+ uptake and their fluxes was recorded and PEPc enzymatic activity in two varieties of Linum usitatissimum. Seeds were germinated under control and saline (100, 200, 250 and 300 mM NaCl) conditions and the effect of salt stress on germination and enzymatic activity was determined. Salinity reduced final germination percentage and lowered germination rate only at concentrations higher than 200 mM, mainly by an osmotic effect. However, NaCl (100 mM) stimulated protein content and PEPc enzymatic activity. These results revealed that the two varieties of Linum usitatissimum are tolerant until 200 mM NaCl.
Keywords: Salinity, tolerance, germination, PEPc, Linum usitatissimum
1. Introduction
Germination is a major factor limiting the establishment of plants under saline conditions (Al-karaki 2001). However, salt stress affects germination percentage, germination rate and seedling growth in different ways depending on plant species. NaCl decreased germination percentage, speed of germination and seedling dry matter in different types of lettuce (Nasri et al. 2011) and reduced final germination percentage in wheat (Almansouri et al. 2001). However, in pepper, NaCl delayed germination but did not reduce the final germination percentage (Chartzoulakis and Klapaki 2000). It is generally believed that most crops are more sensitive to salt during seed germination and seedling growth periods (Cuartero et al. 2006).
In response to salt stress, the plant must develop adaptive mechanisms place to adjust the pressure through the internal osmotic and electrolyte to organic solutes. Malate which synthesis is provided by the PEPC was frequently regarded as one of organic solutes capable of lowering the tissue water potential and improves therefore their hydration (Osmond and Popp 1983). It is commonly accepted that accumulation in the malate cytoplasm used, depending on the species, to offset the massive penetration of essentially of inorganic cations K and Na ions (Whithingten and Smith 1992). Other organic molecules, which appear to play a role in the osmotic cytoplasm under salt stress, have been reported in several species. This is the case of soluble sugars (El Haddad and O'Leary 1994). However, the elements most often associated with plant tolerance to salinity of Protein accumulation commence 8 to 10 h after treatment.
This line shows a high photosynthetic rate (Bandyopadhyay et al. 2007) and increased resistance to oxidative stress (Li et al. 2005). Over-expression of PEPC also relieved the inhibition of photosynthesis under drought stress (Zhou et al. 2010). Other studies showed that PEPC activity and the expression of its encoding gene were related to regulation of stomata in guard cells (Cousins et al. 2007). Phosphoenolpyruvate carboxylase is a cytosolic enzyme in bacteria, cyanobacteria, algae and higher plants. During C4 photosynthesis and Crassulacean acid metabolism (CAM), it plays a cardinal role in the initial fixation of atmospheric CO2, (as HCO3−) into the C4 dicarboxylic acids l-malate and aspartate. Phosphoenolpyruvate carboxylase (PEPC, EC 4.1.1.31), NADP- malic enzyme (NADP-ME, EC 1.1.1.40), and pyruvate, phosphate dikinase (PPDK, EC 2.7.9.1) participate in Hatch-Slack path- way known as C4 photosynthesis. This type of photosynthesis represents one of the CO2 concentrating mechanisms to over- come oxygenase activity of ribulose-1,5-bisphosphate carboxylase/oxygenase (Rubisco) giving advantages to plants especially in warm climate with high irradiance and low water availability (Sage 2004). All these enzymes are also present in C3 plants and non-photosynthetic tissues of all plants; they can be important particularly under stress conditions (Doubnerova and Ryslava 2011).
Proline, as an organic osmoprotectant, largely accumulated in plants subjected to abiotic stresses. In addition to maintaining osmotic balance, proline is also able to stabilize subcellular structures, such as membranes and proteins, quench active oxygen, and protect cells against the adverse effects of salt stress (Ashraf and Foolad 2007). In recent years, considerable amounts of literature have been published about exogenous proline improving the salt tolerance of crops, such as sorghum (Nawaz et al. 2010) rice (Deivanai et al. 2011), canola (Athar et al. 2009) and melon (Kaya et al. 2007)
We suppose that PEPC has an important role in determining the concentration of protein in linum seeds. To test this hypothesis, the PEPC activity of two variety of linum was investigated and the correlations between PEPC activities and final protein, malate/PEPC and Proline/PEPC content of grain were explored.
2.Material and methods
2.1. Plant materials
Linseed (Linum usitatissimum), or flaxseed, is one of the most potent sources of omega 3 fatty acids found in nature. It has a whole host of health benefits, and best of all, these benefits apply to everyone. Linseed improves the quality of hair, nails, and skin, helps to regulate body weight, lowers cholesterol and blood pressure, and prevents arthritis and cancers (Thompson et al. 2005; Zhao et al. 2007).
Two sets of Linum usitatissimum O116 and P126 were used.
2.2. Seed viability and germination speed
The seeds of the linseed used in this investigation were provided by the Seed Laboratory of the Tunisian Ministry of Agriculture. For germination, seeds were soaked for 2 h in distilled water. The seeds were then placed in Petri dishes with double-layer filter paper initially moistened with a solution of the respective salt concentration 0, 100, 200, 250, 300 mM. The Petri dishes were incubated in the dark at room temperature (25 ± 2_C). Each treatment consisted of 25 seeds per Petri dish and was replicated three times. Seeds with emerged radicle were counted daily. Final germination percentage (FG%) was calculated as 100 x number of germinated seeds divided by number of sown seeds. After different periods, seedlings were used for determination of growth parameters. Fresh weights (FW) of all samples were recorded. Plant material was dried at 60_C for 2 days and dry weights (DW) were measured. Tissue water content was obtained from the (FW - DW/FW) ratio. Unless otherwise stated, the same procedure was applied in the following experiments. Time monitoring of germination is 6 days.
2.3. Growth of the radical
Another experiment was conducted to follow the elongation of the radicle of seedlings of two varieties of flax, for 7 days in the presence of different NaCl concentrations (0, 100, 200 and 250 mM NaCl). The concentration of 300 mM NaCl was omitted because the measure of the radicle was not possible at this concentration. The monitoring is done every day at a specific time making photographs using a scanner. Analysis of photographs is made using the Optimas software (version 6.1, Optimas Corporation) (Rodriguez et al. 2002).
2.4. Protein content
Germinating seeds were separately ground in liquid N2, and the obtained powder was resuspended in a 50 mM, pH 7.5 phosphate buffer containing 1 mM EDTA, 1 mM DTT, 5% glycerol and 5% polyvinylpyrrolidone, and centrifuged for 20 min at 15,000 x g. seeds soluble protein content were determined in the supernatant according to the method of Bradford (1976) using bovine serum albumin (BSA) as the standard.
2.5. Enzyme extraction and assay
Fresh seeds were ground in a mortar with 100 mM Tris–bicine (pH 8.0) containing 1 mM EDTA, 5% glycerol (v/v), 5 mM MgCl2, 1% mercaptoethanol (v/v), 1 mM phenylmethylsulfonyl fluoride (PMSF), and 5% polyvinylpyrrolidone (PVP) (w/v of sample FW). After centrifugation at 12,0009g for 20 min at 4C, the supernatant was collected and enzyme activities were measured immediately.
The activities of phosphoenolpyruvate carboxylase (PEPC) and Rubisco were assayed according to Ouerghi et al. (2000). PEPC reaction mixture contained 100 mM Tris–bicine (pH 8.0), 5 mM MgCl2, 1 mM DTT, 5 mM NaHCO3, 0.2mM NADH, 4 mM phosphoenolpyruvate, 5 enzyme units of malate dehydrogenase (MDH). The crude extract (100 µl) was added to the reaction medium then the activity was monitored at 340 nm for 15 min.
2.6. Determination of malate contents
L-malate concentration was determined in aliquots of the seed crude extracts by a spectrophotometric at 340 nm assay in the presence of NAD-dependend MDH and NADH according to Hohorst (1970)
2.7. Proline quantification
The proline content was determined using a modified Bates et al. (1973) method. Powder of tissue (10 mg DM) from leaves was weighed into 1.5 ml centrifuge tubes. The powder was suspended in 1.5 ml of 3% (w/v) sulphosalicylic acid to precipitate protein. Samples were mixed, centrifuged at 14,000 g for 10 min, and the supernatant transferred to a fresh 1.5 ml tube. An aliquot of 1 ml of supernatant was reacted with 1mL of glacial acetic acid and 1ml of ninhydrin reagent (1.25 g ninhydrin in 30 ml glacial acetic and 20 ml phosphoric acid 6M) for 1 h at 100º C before the reaction was stopped by cooling the tubes in ice. The products were extracted with 2 ml of toluene by vortex mixing and the upper (toluene) phase decanted into a glass cuvette and absorbance read at 520 nm. Proline concentrations were calculated from the absorbance of a set of proline standards (0–20 mg ml-1) assayed in an identical manner.
3. Results
Germination kinetics not differed according to varieties but differed according salt treatments (Fig1). All germination curves reached a plateau (FG% final germination percentage), but they differed on the value of FG% (Table 1) and on the time necessary to reach this value.
For O116 seeds, FG% exceeded 95% at 0 and 100 mM NaCl, but it was reduced to ca. 10% and 30% at 200 and 250 mM NaCl. Furthermore, the latency for radicle emergence augmented with salt concentration. In this variety, the germination kinetics at 100 mM NaCl was not distinguishable from that at 0 mM NaCl. The P 129 variety displayed germination kinetics similar to those of O116, except that FG% values were slightly more sensitive to salt at high concentrations (300 mM) and the plateau was reached more slowly. Germination suppressed at 300 mM NaCl. P129 was the most salt-sensitive among the two varieties, with a complete suppression of germination at 300 mM NaCl. In O116 variety, 300 mM NaCl was sufficient to limit FG% at 80% of its value at 0 mM NaCl.
|
Figure 1: Evolution of germination percentage of two Linum usitatissimum varieties (O116 and P129) under different NaCl treatments (0, 100, 200, 250 andt 300 mM). Data are the mean of three samples of 25 seedlings each one |
Table 1: Effect of different NaCl treatments on the final germination percentage %, latency time and constant of speed of two Linum usitatissimum varieties. |
|
The effect of salt on the elongation of the radicle of seedlings of two varieties of flaxseed is shown in table 2. It shows that the salt affects the growth of the radicle length of seedlings in both varieties of flax. This reduction is even more important than the NaCl concentration is higher. After 168 hours and a concentration of 100 mM NaCl, the reduction is 30% and 40% compared to the control, respectively, in seedlings of the variety Ø116 and those of the P129 range. At 200 mM NaCl, this reduction is about 70% of the radicle of both varieties (Ø116 and P129). At 250 mM NaCl, the length of the radicle is reduced by 85% in seedlings of two varieties (Ø116 and P129). At 300 mM NaCl, only the variety of seeds to germinate but Ø116 arrive, their radicles have lengths weak. Therefore, radicle growth was more affected by salt than was shoot growth. Radicle elongation was still more sensitive to salt inhibition: radicle length was diminished by more than 80% (O116 and P129) by salt 250 mM.
O116 (the variety with the most salt tolerant germination) and P129 (moderately salt sensitive germination) were chosen to analyze the effect of salt on enzymatic activities and protein content at different concentrations of NaCl. Obtained results in figure 2 (protein) shows that, during first days in germination under salt stress, protein synthesis was found to be important in O116 species. It may be that sufficient cellular protection had occurred during imbibition and first day in germination. The signature response to salt stress in a decrease in the synthesis of normal protein.
Table2: Effect of different NaCl treatments on radicle length in two varieties of Linum usitatissimum SEEDS (O116 and P129) grown under salt stress condition. |
|
Developmental profiles for seed PEPC activity are shown in figure 3. In Both varieties, PEPC activity sharply increased during germination. A marked difference was observed Between the Two varieties, PEPC activity was very low during the first days of germining, followed by a spectacular Increase, so that the maximum activity of PEPC corresponded to the fourth days of germination for the control. An increase in the activity of this enzyme is observed upon salt treatment, the maximum activity of PEPC was recorded at the third day of seed germination in O116 and fourth day in P126 to 250 mM NaCl.
The K:Na, malate and proline concentration recorded in germination of two linseeds varieties (Table 3). Data of K+ and Na+ fluxes is presented in table 3. Greater fluxes of K and less of Na were observed for two seeds varieties at the higher NaCl concentration (100 mM). Malate and Proline contents were increased with salinity but did not correlate with the salinity tolerance (Table.1). Salt stress increased the concentrations of proline in two varieties but there was considerable variation in the amount of proline observed among the linseeds varieties in response to salinity.
Table 3: Mean sodium, potassium concentration, malate and proline concentration (mg g-1 dry weight) in Seed germination of two varieties under 0 and 100 mM NaCl salinity. |
|
4. Discussion
Germination constitutes a precocious test for researching threshold plants tolerance to salt stress. Studying linseeds behaviour, O116 and P126 cultivars, during germination at different concentrations of NaCl (Fig. 1) in comparison with control (0 mM NaCl) reveales that salt stress reduced germination capacity and delayed germination. However, high concentration of NaCl affected germination capacity and increased lightly the delay time. The prolonged delay time want to prevent radicle protrusion and defended to obtain seedlings enable to grow properly. These species have an aptitude to germinate at 200 mM NaCl. The nature of adaptation process and the intensity of the response vary among plants species and among the different cultivars.
Given the results of this study, it appeared that salt treatment slowered down germination almost in the same way, in two varieties (Fig 1). It reduced significantly the final germination percentage (FG% max) since the lower NaCl concentration (200 mM) in two varieties, and only at the higher NaCl concentration (300 mM) in O116 (Table 1). In accordance with our results Nasri et al. (2011) reported that germination of four lettuce varieties decreased as the salinity concentration increased and salinity also delayed germination rate. Differences of salt sensitivity, at the germination stage, were also described by Jamil and Rha (2004) in sugar beet and Cabbage under saline conditions, FG%max was reduced in beet and Cabbage cultivars. On the whole, these results indicated that increased salinity results in decrease in germination percentage and rate of germination (Almansouri et al. 2001).
Seed germination of linseeds as observed in this study, generally decreases with increasing salinity and most un-germinated seeds of test species recovered completely when transferred to distilled water indicating osmotic rather than ionic effects (Gul et al. 2013). Osmotic effects of salinity on seed germination were also reported for A. halimus (Bajji et al. 2002). Lower seed germination with an increase in salinity in our study was perhaps due to decrease in water uptake as in some other plants (Song et al. 2005). According to Al-Karaki (2001) the adverse effect of salt stress on seed germination in barley may result from internal osmotic stress or restricted imbibitions rather than from ion toxicity effects.
Data of K+ and Na+ fluxes is presented in table 3. Greater fluxes of K and less of Na were observed for two seeds varieties at the higher NaCl concentration (100 mM). According to Poustini and Siosemardeh (2004) the monocots salinity tolerance is associated with the ability of plant to exclude Na+ and maintain high K/Na in shoot tissues. In wheat genotypic variation in Na+ and K+ uptake has already been reported (Munns 2007). Enhanced leaf K/Na ratio and greater fluxes of K and less of Na are the factors determining salt tolerance in wheat (Poustini and Siosemardeh 2004; Munns 2007). Salt stress increased the concentrations of proline in two varieties but there was considerable variation in the amount of proline observed among the linseeds varieties in response to salinity.
It is obvious that the germination capacity may be reduced by the effects of both osmotic and toxic (Abdelly 1997). Indeed, Figure 4 shows that the percentage of seed germination of two varieties of linseed is kept constant and close to 100% to a value of osmotic potential of the imbibition solution equal to -4.89105 Pa. in addition, the germination percentage decreases to a value of 15% for the variety and Ø116 zero in seeds of the variety P129, with a value equal to the osmotic potential -7.33105 Pa.
For osmotic adjustment the accumulation of ions is more favourable in terms of energy cost. However, under osmotic stress many plants also accumulate organic osmolytes such as proline and glycinebetaine (Shafi et al. 2011). Compatible organic solutes are low molecular weight, non-toxic water soluble organic molecules also known as osmoprotectants (Chen and Murata 2002) that play role in osmotic adjustment. Plants accumulate free proline to maintain osmotic adjustment under salinity stress (Farouk 2011). It not only acts as an indicator of salt tolerance but also indicate a sign of reaction to salt induced damage (Carpici et al. 2010) stabilizing membranes integrity, enzymes regulation and detoxification of ROS in plants under salt stress (Misra and Saxena 2009). Maintain cell turgidity or relative water contents effectively (Hossain et al. 2011). Proline synthesis under salt stress might be due to increased synthesis of proline synthesizing enzymes or decreased activity of proline degrading enzymes (Misra and Saxena 2009). Proline is among most frequently occurring compounds that play key role in osmotic adjustment (Ueda et al. 2007). In wheat proline acts as an endogenous osmotic regulator and its level in plant tissues indicates plants ability to adapt to environmental conditions especially salt stress (Munns et al. 2006).
As shown in literature, plants and organisms respond to high concentration of NaCl with the induction of osmotique shock proteins. Proteins are postulated to protect organisms from the damaging effects of salt and others forms of stress.
Obtained results in Figure 4 show that, at 100 mM NaCl, there is not a markedly change in proteins content during germination, mainly at first 32 h. At this stage, a slight increase in protein amount was observed in control condition (0 mM). During imbibition (first eight hours) under high salinity (200 and 250 mM NaCl), protein synthesis was found to be important in O116 and P129 species. It may be that sufficient cellular protection had occurred during imbibition (8 h) at 250 mM NaCl.
|
Figure 4: relationship between germination percentage and osmotic potential in two varieties of Linum usitatissimum SEEDS (O116 and P129) grown under salt stress condition |
Differently with our results, Dell' Aquila and Spada (1992) says in both genotypes of wheat, At 72 h the synthesis of salt-induced proteins persisted with no significant change in Norba embryos while it was reduced in the 7675 embryos, where proteins associated with the radicle emergence phase were produced to water after 48 h of salinity stress, the synthesis of proteins specific to radicle emergence and growth phase was resumed, but salt-induced proteins ceased to be synthesized .
Seed germination is an appropriate stage in the life of plants to analyse differences in gene expression induced by salinity. When the normal hydration of wheat seeds is interrupted by salt stress, the complex process of radicle emergence is blocked and the biochemical mechanisms involved can be impaired (Dell' Aquila and Spada 1992). Protein synthesis changes are due to changes in the efficiency of mRNA translation or to regulation of mRNA transcription, transport and stability. As a wheat embryo passes from a quiescent to a proliferative stage, considerable changes in mRNAs occur, including a rapid loss of mRNAs coding for storage proteins (Cuming 1984), and the appearance of mRNAs specific for new proteins associated with the germination process (Bewley and Marcus 1990).
Dell' Aquila and Spada 1992 show that the primary effect of salinity is the repression of specific groups of proteins associated with an advanced germination stage and a differential qualitative expression of a small fraction of newly-made polypeptides in both cultivars. Moreover in the salt-tolerant genotype the synthesis of some salt-induced proteins common to the salt- sensitive genotypes increases before radicle emergence and subsequently is reduced at the onset of germination in the saline solution. The accumulation of these specific proteins, not produced during water imbibition, suggests that the function of' salt stress' proteins may be related to the seed adapting to salinity.
Our results show that in both varieties, PEPc activity increased during germination. A marked difference was observed Between the Two varieties, PEPc activity was very low during the first days of germining, followed by a spectacular Increase, so that the maximum activity of PEPc corresponded to the fourth days of germination for the control. An increase in the activity of this enzyme is observed upon salt treatment, the maximum activity of PEPc was recorded at the third day of seed germination in O116 and fourth day in P126 to 250 mM NaCl (fig 3). Increased PEPC activity, was also described by Amdouni et al. (2014) in mais plant under saline conditions. Also, PEPC activity increases during germination of Ricinus communis (Podesta and PIaxton 1994a) and Sorghum seeds (Khayat et al., 1991). In wheat seeds (aleurone-endosperm), results show that total PEPC activity is relatively constant, but the specific activity increases about 50% during the first 48 h of imbibition and then decreases slightly, thereby indicating the general stability of this protein during germination. In vivo, the enzyme is subjected to interacting metabolic (malate, feedback inhibitor, and glucose-6-phosphate, allosteric activator) and covalent (phosphorylation) controls that modulate its activity in relation to the physiological context (Vidal et al. 1997). In seeds, PEPC is found in most tissues and the embryo (Gonzalez et al. 1998) and it has been postulated that it is involved in the synthesis of storage compounds.
Major PEPC functions include C4 and CAM photosynthesis and the anaplerotic pathway leading to amino acid synthesis. A similar function has been ascribed to the PEPC of germinating maize and sorghum seeds (Khayat et al, 1991). To find the relationship between the activity of PEPC and synthesis of proline and malate, we determined the correlation coefficients between the two. The results (Table 4) show that there is a close correlation between the activity of PEPC and synthesis of proline and malate. Similarly, the difference in the degree of accumulation of proline and leaf malate in both durum wheat genotypes reveals a close relationship between salt tolerance and accumulation capacity of these two elements (Lidia Osuna et al. 1996).
Table 4: Correlation coefficients between proline / PEPC and malate / PEPC in two varieties of Linum usitatissimum Seeds (O116 and P129) grown under salt stress condition |
|
It seems that whenever an increase in PEPC activity is required in a physiological context, the malate pool is expected to increase and, thus, PEPC needs enhanced protection (phosphorylation) against this feedback inhibitor for the carboxylation reaction to proceed efficiently in these adverse conditions. PEPC activity in wheat seed extracts was found to be subject to metabolite regulation.
The present results pave the way for studies on the purification and determination of the functional properties of PEPCs and PEPC kinase(s) from linum seeds. In addition, it should be investigated whether a signal transduction chain controls the phosphorylation of PEPC during both germination and maturation of seeds.
5. References
Abdelly C (1997) Mécanismes d’une association de luzernes spontanées et de halophytes pérennes en bordure de sebkha. Thèse Doc. Etat FST, Univ Tunis II.
Al-Karaki GN (2001). Germination, sodium and potassium concentrations of barley seeds as influenced by salinity. J Plant Nutr 24: 511
Almansouri M, Kinet JM, Lutts S (2001) Effect of salt and osmotic stresses on germination in durum wheat (Triticum durum Desf.). Plant Soil 231: 243
Amdouni T, M’rah S, Msilini N, Zaghdoudi M, Ouerghiabidi Z, M. Lachaal (2014). Physiological and biochemical responses of two maize cultivars (Corralejo and Tlaltizapon) under salt stress. Journal of Stress Physiology & Biochemistry, 10 (3): 246-258
Ashraf M, Foolad MR (2007) Roles of glycine betaine and proline in improving plant abiotic stress resistance. Environ Exp Bot 59(2): 206.Athar H U R, Ashraf M, Wahid A et al (2009) Inducing salt tolerance in Canola (Bras sica Napus L) by exogenous application of glycinebetaine and proline: response at the initial growth stages. Pak J Bot 41(3): 1311.
Bajji M, Kinet JM, Lutts S (2002) Osmotic and ionic effects of NaCl on germination, early seedling growth, and ion content of Atriplex halimus (Chenopodiaceae). Can J Bot 80: 297.
Bandyopadhyay A, Datta K, Zhang J, Yang W, Raychaudhuri S, Miyao M (2007) Enhanced photosynthesis rate in genetically engineered indica rice expressing PEPC gene cloned from maize. Plant Sci 172 (6): 1204.
Bates LS, Waldren RP, Teare ID (1973) Rapid determination of free proline for water-stress studies. Plant Soil 39: 205
Bewley JD, Marcus A (1990) Gene expression in seed development and germination.
In: Cohn WE, Moldave K eds. Progress in nucleic acid research and molecular biology. 38. San Diago Academic Press 165.
Bradford MM (1976) A Rapid and Sensitive Method for the Quantitation of Microgram Quantities of Protein Utilizing the Principle of Protein-Dye. Binding Anal Biochem 72:248.
Carpici EB, Celik N, Bayram G, Asik BB (2010) The effects of salt stress on the growth, biochemical parameter and mineral element content of some maize (Zea mays L.) cultivars. Afr J Biotechnol 9(41): 6937.
Chartzoulakis K, Klapaki G (2000) Response of two greenhouse pepper hybrids to NaCl salinity during different growth stages. Sci Hortic 86: 247
Chen TH, Murata N (2002) Enhancement of tolerance of abiotic stress by metabolic engineering of betaines and other compatible solutes. Curr Opin Plant Biol 5: 250.
Coming AC (1984) Developmental regulation of gene expression in wheat embryos. Molecular cloning of a DNA sequence encoding the early methionine-labelled (Em) polypeptide. Euro J Biochem 145: 351.
Cousins AB, Baroli I, Badger MR, Ivakov A, Lea PJ, Leegood RC, Caemmerer SV (2007) The role of phosphoenolpyruvate carboxylase during C4 photosynthetic isotope exchange and stomatal conductance. Plant Physiol 145: 1006.
Cuartero J, Bolarin MC, Asins MJ, Moreno V (2006) Increasing salt tolerance in the tomato. J Exp Bot 57(5): 1045.
Deivanai S, Xavier R, Vinod et al (2011) Role of exogenous proline in ameliorating salt stress at early stage in two rice cultivars. J Stress Physiol Biochem 7(4): 157-174.
Dell’Aquila A, Spada P (1992) The Effect of Salinity Stress upon Protein Synthesis of Germinating Wheat Embryos. Annals of Botany 72: 97.
Doubnerova V, Ryslava H (2011) What can enzymes of C4 photosynthesis do for C3 plants under stress. Plant Sci180: 575.
Farouk S (2011) Ascorbic acid & α-tocopherol minimize salt-Induced wheat leaf senescence. J Stress Physiol Biochem 7: 58.
Gonza´lez MC, Osuna L, Echevarr´ıa C, Vidal F. Cejudo J (1998) Expression and localization of phosphoenolpyruvate carboxylase i J.n developing and germinating wheat grains. Plant Physiol 116: 1249.
Gul B, Ansari R, Flowers TJ, Khan MA (2013) Germination strategies of halophyte seeds under salinity. Environ Exp Bot 91: 22.
Hohorst HJ (1970) L-malate estimation with malate dehydrogenase and NAD, in: H.V. Bergmeyer (Ed.), Methods in Enzymatic Analysis, Verlag Chemie, Weinheim 1544.
Hossain M, Hasanuzzaman M, Fujita M (2011) Coordinate induction of antioxidant defense and glyoxalase system by exogenous proline and glycinebetaine is correlated with salt tolerance in mung bean. Front Agric China 5: 1.
Jamil M, Rha ES (2004) The effect of salinity (NaCl) on the germination and seedling of sugar beet (Beta vulgaris L.) and cabbage (Brassica oleracea L.). Korean J. Plant Res 7:226.
Kaya C, Tuna AL, Ashraf M, Altunlu H (2007) Improved salt tolerance of melon (Cucumis melo L.) by the addition of proline and potassium nitrate. Enviro Exp Bot 60(3): 397.
Khayat E, Dumbroff EB,Glick BR (1991) The synthesis of phosphoenolpyruvate carboxylase in imbibing Sorghum seeds. Biochem Cell Biol 69: 141.
Li X, Jiao DM, Dai CC (2005) The response to photooxidation in leaves of PEPC transgenic rice plant (Oryza sativa L.). Acta Agron Sin 31:408.
Mishra V, Saxena DK, Das M (2009) Effect of argemone oil and argemone alkaloid, sanguinarine on Sertoli-germ cell coculture. Toxicol Lett 86(2):104.
Munns R (2007) Utilizing genetic resources to enhance productivity of salt-prone land.Perspect in Agri.Veter. Sci. Nut and Natural Resources. 2:11.
Munns R, James RA, Lauchli A (2006) Approaches to increasing the salt tolerance of wheat and other cereals. J Exp Bot 57: 1025-1043
Nasri N, Kaddour R, Rabhi M, Plassard C, Laachal M (2011) Effect of salinity on germination, phytase activity and phytate content in lettuce seedling. Acta physiol Plant 33: 935.
Nawaz K, Talat A, Iqra K, Hussain, Majeed A (2010) Induction of salt tolerance in two cultivars of sorghum (Sorghum bicolor L.) by exogenous application of proline at seedling stage. World Appl Sci J 10(1): 93.
Nimmo HG (2003) Control of the phosphorylation of phosphoenolpyruvate carboxylase in higher plants. Arch Biochem Biophys 414: 189.
Osmond CB, Popp M (1983) The balance of malate synthesis and metabolism in response to ion uptake in excised wheat roots. Plant Sci Letters 32 : 115.
Osuna L, Gonzalez MC, Francisco, Cejudo J, Vidal J, Echevarria C (1996) In Vivo and in Vitro Phosphorylation of the Phosphoenolpyruvate Carboxylase from Wheat Seeds during Germination. Plant Physio 111: 551.
Ouerghi Z, Cornic G, Roudani A, Ayadi A, Brulfert J (2000) Effect of NaCl on photosynthesis of two wheat species (Triticum durum and T. aestivum) differing in their sensitivity to salt stress. J Plant Physiol 156: 335.
Podesta FE, Plaxton WC (1994a). Regulation of cytosolic carbon metabolism in germinating Ricinus communis cotyledons. I. Developmental profiles for the activity, concentration, and molecular structure of the pyrophosphate and AIP-dependent phosphofructokinases, phosphoenolpyruvate caboxylase and pyruvate kinase. Planta 194: 374.
Poustini K, Siosemardeh A (2004) Ion distribution in wheat cultivars in response to salinity stress. Field Crops Research 85(2-3):125.
Rodríguez AA, Grunberg KA, Taleisnik EL (2002) Reactive Oxygen Species in the Elongation Zone of Maize Leaves Are Necessary for Leaf Extension1 Plant Physiol. 129(4): 1627–1632.
Sage RF (2004) The evolution of C4 photosynthesis. New Phytologist 161: 341.
Shafi M, Bakht J, Khan MJ, Khan MA, Din MR (2011b) Role of abscisic acid and proline in salinity tolerance of wheat genotypes. Pak J Bot 43: 1111.
Song, J, Feng G, Tian C, Zhang F ( 2005) Strategies for adaptation of Suaeda physophora, Haloxylon ammodendron and Haloxylon persicum to a saline environment during seed germination stage. Ann Bot (London) 96: 399.
Thompson LU, Chen JM, Li T, Straaser WK, Goss PE (2005) Dietary flaxseed alters tumor biological markers in post menopausal breast cancer. Clin. Cancer Res. 11: 3828-3835.
Ueda A, Yamamoto-Yamane Y, Takabe T (2007) Salt stress enhances proline utilization in the apical region of barley roots. Biochem Biophy Research Com 355: 61.
Ullah SM, Soja G, Gerzabec MH (1994) Ion Uptake osmoregulation and plant water relations in fababeans (Vicia faba) under salt stress. J Fûr Landwirtschaft Lich Forschung 291.
Vidal J, Chollet R (1997) Regulatory phosphorylation of phosphoenolpyruvate carboxylase. Trends Plant Sci 2: 230.
Whittington J, Smith FA (1992) Salinity induced malate accumulation in Chara. J Exp Bot 43 (251): 837.
Zhao G, Etherton TD, Martin KR, Gillies PJ, West SG, Kris-Etherton PM (2007) Dietary α- linolenic acid inhibits proinflammatory cytokine production by peripheral blood mononuclear cells in hypercholesterolemic subjects. Am. J. Clin. Nutr., 85(2): 385-391.
Zhou BY, Ding ZS, Zhao M (2010) Alleviation of drought stress inhibition on photosynthesis by overexpression of PEPC gene in rice. Acta Agron Sin. 37:112